|
|
|
The Chemist Volume 94 | Number 1 |

|
|
Surface Chemistry and Biomolecule Density Impact Adsorbed Cellulase Activity
|
|
Abstract: Bioethanol produced from inedible biomass has the potential to curtail emissions, maximize usage of agricultural/urban waste and be a renewable energy source. Hydrolyzing cellulose to glucose is a critical step in the production of bioethanol from inedible biomass and can be accomplished through the use of enzymes, cellulases, which hydrolyze cellulose into sugars that are subsequently fermented into ethanol. To diminish the economic impact cellulases impart on the production of bioethanol, cellulases can be immobilized to magnetic nanoparticles enabling their separation and reuse. The functional group and biomolecule density on nanoparticles are intertwining variables that affect the specific activity of immobilized enzymes, a pivotal parameter that need be maximized for an industrial catalyst. This work presents the findings of how functional group and biomolecule density on silica-coated iron oxide nanoparticles relate to the activity of adsorbed cellulases. The adsorption percent correlated to increasing functional group density, while the specific activity was inversely proportional to functional group density. At high functional group densities, the increase in immobilization led to a greater recovery of activity. The results conclude that adsorbing cellulase from dilute solutions to particles with high functional group densities displayed the greatest efficiency for adsorbing cellulases.
Key Words: cellulase, immobilized enzymes, nonspecific absorption, cellulosic ethanol
|
|
|
Introduction
There is a critical need to develop sustainable energy sources. Lignocellulose is the most abundant renewable resource and is ~45% cellulose, a polymer of D-glucose linked by β-1,4-glycosidic bonds [1]. Bioethanol derived from cellulose can be a sustainable energy source but requires extensive processing to hydrolyze the cellulose into glucose prior to fermentation into bioethanol. Cellulose hydrolysis can be performed through chemical or enzymatic methods to yield fermentable sugars. Enzymatic hydrolysis of cellulose, using cellulases, has the advantage over non-enzymatic methods as the cellulases operate at gentle temperatures, meaning enzymatic hydrolysis necessitates a lower energy input compared to alternative chemical hydrolysis methods. However, cellulases contribute an appreciable cost to the production of bioethanol which has hindered widespread adoption of enzymatic hydrolysis of cellulose [2,3]. Therefore, lowering the economic cost of cellulases will lead to commercialization of bioethanol from cellulose.
Immobilizing enzymes on solid supports facilitates their separation from product and enables reuse of the enzyme. The chemistry of the support material and method of biomolecule immobilization affect the properties of the immobilized catalyst. There are several types of support being studied for cellulase immobilization such as metal-organic frameworks [4], organic supports [5], and inorganic supports [6]. However, magnetic nanoparticle (MNP) supports facilitate rapid recovery of immobilized catalyst by application of an external magnetic field. The ease with which MNPs can be separated and reused has led to their study as support for cellulase immobilization [7]. The most routine methods of cellulase immobilization are adsorption, covalent attachment, entrapment, and crosslinking [8]. Each method of immobilization offers unique advantages, but adsorption is the simplest method requiring few reagents or steps.
Adsorption relies on nonspecific interactions such as hydrophobic, electrostatic, and/or Van der Waals forces between the enzyme and support. Adsorption, while simple, may lead to deleterious conformational changes of the immobilized biomolecule altering biomolecule activity. Some command over the adsorption process is obtained by controlling the chemistry of the support surface. Amine functional groups are routinely introduced to supports to enhance electrostatic interactions between the support and biomolecule to promote adsorption. A common regent utilized to introduce amine functionality to MNPs is (3-aminopropyl)triethoxysilane (APTES) [9]. Varying the APTES concentration during modification can be used to introduce varying degrees of amine functional groups [10], which affects immobilized biomolecule densities.
The concentration of the biomolecule solution utilized for immobilization also effects adsorption, with increased adsorption occurring from concentrated biomolecule solutions [11] as the bulk concentration determines the kinetics of the immobilizations process [12]. Concentrated biomolecule solutions will have biomolecules adsorb quickly with close proximity to one another, and lead to a dense protein layer. Slower adsorption kinetics allows initially adsorbed biomolecules time to undergo conformational changes to maximize interactions with the surface. Biomolecules that “spread out” to maximize interactions with the particle surface prevent adsorption by later biomolecules and lower biomolecule density occurs when adsorbed from dilute solutions [12,13].
The surface chemistry and bulk biomolecule concentration jointly determine the adsorption process which determine immobilized quantities and may lead to altered biomolecule activity. Reduced activity is observed at high immobilized biomolecule densities due to steric hindrance imposed by adjacent molecules or by conformational changes at low densities as the biomolecule maximizes contact with the surface [14,15]. It is therefore of great consequence to consider both the bulk biomolecule concentration used for adsorption, and the surface functional group density jointly when optimizing an immobilization method to ensure the support adsorbs an abundance of biomolecules in an active conformation.
Each biomolecule is unique, as will be its interactions with the support [16]. Therefore, the immobilization conditions need be optimized for each biomolecule. In this work MNPs with varying densities of APTES were synthesized and adsorbed with cellulases from two different bulk concentrations. MNPs reacted with high degrees of APTES had increased cellulase adsorption levels, lower specific activities, but higher activity recovery. The cellulase adsorbed to MNPs from dilute bulk solutions had markedly higher immobilization efficiencies, and specific activities, expressing a 4 to 30-fold enhancement in specific activity relative to the free enzyme. These results express the importance in adsorbing cellulases from dilute bulk solutions to MNPs with high APTES densities to ensure efficient immobilization and recovery of cellulase activity.
Materials and Methods
Iron (III) chloride hexahydrate (FeCl3∙6H2O) and glutaraldehyde (50%) were purchased from Sigma-Aldrich (St. Louis, MO, USA). Iron (II) chloride tetrahydrate (FeCl2∙4H2O), and 3-aminopropyltriethoxysilane (APTES) were purchased from Acros Organics (Geel, Belgium). Cellulase from T. reesei was purchased from Abnova (Walnut, CA). Copper (II) sulfate pentahydrate (CuSO4∙5H2O), sodium acetate, sodium carbonate, sodium bicarbonate, sodium monohydrogen phosphate, sodium dihydrogen phosphate, ammonium hydroxide (29%) and tetraethyl orthosilicate (TEOS) were purchased from Fisher Scientific (Pittsburgh, PA). Sodium bicinchoninate (BCA), carboxymethyl cellulose sodium salt n ~ 500 (CMC) was purchased from Tokyo Chemical Industry (Portland, USA). The 3,5-dinitrosalicylic acid (DNS) was purchased from Spectrum (New Brunswick, NJ).
A 47 mL solution containing 60 and 30 mM of Fe3 and Fe2+, respectively, in 18 MΩ H2O was heated to 80°C and degassed with N2. After 30 min of degassing, 2.2 mL of NH4OH was added dropwise and allowed to react for 1 hr. The black product was washed using degassed 18 MΩ H2O three times followed by three subsequent washes with ethanol. The volume was brought to 53 mL with ethanol and 2 mL of 200 mM TEOS was added. The hydrolysis of TEOS was initiated by the addition of 5 mL NH4OH. The solution was placed on a shaker set at 25°C and 350 RPM overnight.
The amine functional groups were introduced to the MNPs by the condensation of APTES. The TEOS modified MNPs were washed with ethanol three times and 30 mg of MNP was added to a scintillation vial containing 10 mL of 3-300 mM APTES in ethanol. The vials were placed on a shaker set at 25°C and 350 RPM for 12 hrs. After amine modification, the MNPs were washed three times with ethanol and dried in a vacuum oven at 60°C overnight.
A ninhydrin assay was performed to determine the surface available amines on the APTES modified particles [17]. In brief, 1.5 mg of MNP was dispersed in 500 µL of 60% ethanol and 100 µl of 20 µM KCN in pyridine, 75 µL of 80% (v/v) phenol in ethanol, and 75 µL of 0.5% ninhydrin in ethanol were added. The solutions were heated to 98°C for 15 min, cooled to room temperature. MNPs were magnetically separated from the supernatant. The absorbance of the supernatants was measured at 570 nm and compared to a hexylamine standard.
The MNPs were dispersed in 10 mM acetate buffer pH 5.0 at a concentration of 2 mg/mL. Cellulase in acetate buffer was added to the MNPs at a 1:1 or 0.1:1 mass ratio of cellulase to MNP. The 1:1 and 0.1:1 cellulase:MNP mass ratios are subsequently referred to as either the high or low loadings, respectively. The MNP/cellulase solutions were placed on a shaker set at 25 °C and 350 RPM for 2 hrs, after which the cellulase immobilized MNPs were washed with acetate buffer three times. The supernatant from each wash was collected and analyzed by the BCA assay using BSA as a standard. The amount of cellulase immobilized was determined by mass balance and the immobilization percent was determined according to equation 1. where Ci is the initial concentration of the cellulase solution, Vi is the initial volume of solution, Cs is the concentration of the supernatant and Vs is the volume of the supernatant.

The DNS assay was used to determine the activity of the cellulase immobilized MNPs using glucose as a standard. The DNS solution contained 27 mM DNS, 0.5 M NaOH, 0.64 M sodium potassium tartrate, and 25 mM sodium metabisulfite. A total of 200 µL of 0.8% (w/v) CMC in 10 mM acetate pH 5.0 was added to 200 µL of cellulase adsorbed MNPs or free cellulase in 10 mM acetate pH 5.0. The samples were placed on a shaker at 50°C for 30 min, after which 200 µL of supernatant was added to 500 µL of DNS solution and boiled for 5 min. The boiled samples were cooled to room temperature and their absorbance measured at 540 nm.
Results and Discussion
MNPs were synthesized and reacted with varying APTES/Fe mole ratios. APTES contains a single primary amine, and the solvent exposed amine density on the MNPs was determined by the ninhydrin assay. The surface amine density correlated to the APTES reactant mole ratio as displayed in Figure 1.
However, the greatest surface amine density was not observed at the highest APTES/Fe mole ratio. APTES is capable of forming a multilayer on a surface [18]. The ninhydrin assay quantifies solvent accessible amines [17] and is insensitive to amines buried in a multilayer or amines that are inaccessible to solvent. The decrease in solvent exposed amines on the MNPs at the highest APTES density is therefore interpreted as the development of an APTES structures that have an inaccessible amine group.
Cellulase was adsorbed to the modified MNPs in 10 mM acetate pH 5.0 for 2 hrs incubated at 25°C. The amount of cellulase immobilized correlated to the cellulase bulk concentration and APTES density, while the immobilization efficiency was dependent upon the APTES density as shown in Figure 2.
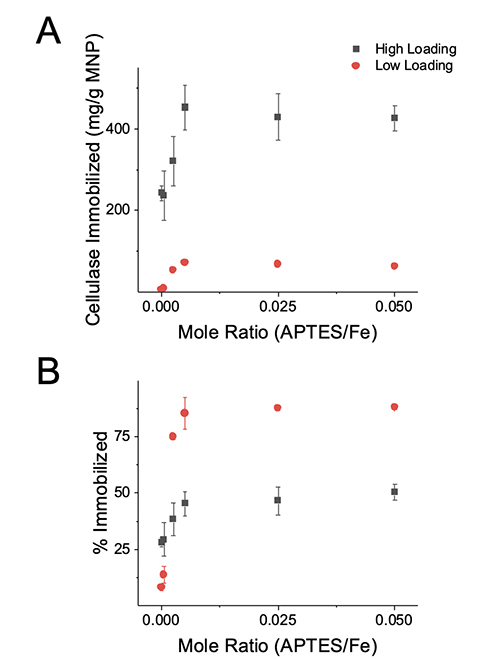 |
The results were anticipated as increasing the APTES density and bulk protein concentration shift the equilibria to favor adsorption of additional biomolecules. The MNPs appear to approach saturation with cellulase at 70 and 450 mg/g MNP for the low and high loadings, respectively. The difference in saturation values between the two loadings may be caused by different surface structures governed by different adsorption processes between the different loadings. At high biomolecule loadings, a greater saturation value is expected as adsorbed biomolecules form a densely packed structures or form multilayers [11,19]. At low biomolecule loadings, a lower saturation value may be caused by adsorbed biomolecule “spreading out” to maximize favorable interactions with the surface, thereby preventing adjacent biomolecule adsorption on the surface [11]. To elucidate whether adsorbed biomolecules suffered deleterious conformational changes or steric hindrance from adjacent biomolecules, the activity of MNPs were assessed.
The DNS assay was used to determine the activity of the MNPs in 10 mM acetate pH 5.0 at 50°C for 30 min using CMC as a substrate. The activity was normalized to the dry mass of MNPs and referred to as particle activity. The particle activity correlated to MNPs to the quantity of cellulase adsorbed as displayed in Figure 3A. However, the
amount of cellulase adsorbed did not linearly correlate with particle activity, as a sixfold increase in cellulase adsorption at the high loading, relative to the low loading, led to only a twofold increase in particle activity. The nonlinear relationship between adsorbed biomolecule quantity and particle activity implies a change in specific activity.
To examine the changes in specific activity, the particle activity was normalized to the amount of enzyme immobilized. The specific activity was inversely proportional to both the loading density and APTES density as shown in Figure 3B.
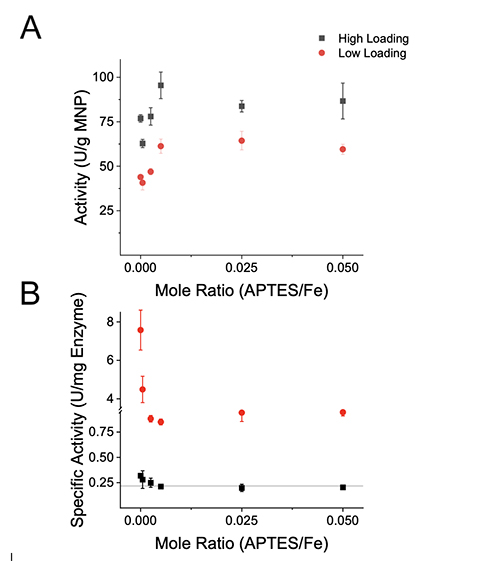 |
Interestingly, the immobilized cellulase had up to a thirtyfold enhancement in specific activity relative to the free enzyme. The immobilized cellulase specific activity was inversely related to the APTES density, which ranged from 0.85 to 7.6 U/mg at the low loading and is significantly different from the free enzyme specific activity of 0.218 ± 0.002 U/mg at the 99% confidence interval as determined by a one-way ANOVA with a TUKEY HSD post-hoc test. The enhanced specific activity could be attributed to favorable conformational changes or an immobilized geometry that favors interaction with the substrate. Immobilized lipase exhibits up to a twentyfold hyperactivity as the immobilized form adopts an open conformation with an exposed active site [20]. The surface structure of the adsorbed biomolecules in this work is unknown. However, it is interpreted that the biomolecules are crowded at the high loading and high APTES densities and, as a consequence, have reduced specific activities. Crowding of biomolecules would restrict their ability to interact with substrate and/or restrict biomolecule mobility needed for catalysis causing a reduction in specific activity.
To quantitatively assess how the bulk cellulase concentration and particle APTES density intertwine to determine the efficiency of the adsorption process, the recovered cellulase activity for each sample was calculated. The units of immobilized activity were normalized to the units of activity used during adsorption to calculate the recovered activity as displayed in Figure 4.
 |
The heightened specific activity and immobilization percentage observed at the low loading led to recovered activities ranging from 127 to 163%, while the high loading had recovered activities ranging from 36 to 47%. The recovered activities illustrate that increasing the APTES functional groups on a surface may be detrimental to the specific activity of immobilized biomolecules, but at high APTES densities a greater recovery of enzymatic activity occurs stemming from the enhanced immobilization percentage. The result from this study indicates increasing the amount of APTES adhered to the surface of the iron nanoparticle will lead to the efficient recovery of catalyst activity, ultimately leading to lower costs of bioethanol produced from cellulose.
Conclusion
In the present work, the recovered activity and immobilization efficiency of cellulases adsorbed to magnetic nanoparticles were explored. The APTES functional group density and bulk biomolecule concentration used during immobilization were inversely proportional to the adsorbed biomolecule activity. However, the immobilization efficiency increased with APTES density, which led to a greater recovery of immobilized activity at high APTES densities. These results indicate a promising future for cellulosic ethanol production by immobilizing cellulases from dilute solutions onto MNPs with high functional group densities to ensure an abundance of cellulase is immobilized in an active conformation.
Acknowledgment
Funded by The Citadel’s Near Center for Climate Studies through a Climatological Research Studies Grant.
References
- Bhaumik P, Dhepe PL in Biomass Sugars for Non-Fuel Applications, The Royal Society of Chemistry, 2016, ch. 1, pp 1-53.
- Sinitsyn AP, Sinitsyna, OA. Bioconversion of renewable plant biomass. Second-generation biofuels: Raw materials, biomass pretreatment, enzymes, processes, and cost analysis. Biochemistry (Moscow), 2021, 86 (1), S166-S195.
- Liu G, Zhang J, Bao J. Cost evaluation of cellulase enzyme for industrial-scale cellulosic ethanol production based on rigorous aspen plus modeling. Bioprocess Biosyst. Eng., 2016, 39(1), 133-140.
- Ahmed IN, Yang X-L, Dubale AA, Li R-F, Ma Y-M, Wang L-M, Hou G-H, Guan R-F, Xie M-H. Hydrolysis of cellulose using cellulase physically immobilized on highly stable zirconium based metal-organic frameworks. Bioresour. Technol., 2018, 270, 377-382.
- Ho KM, Mao X, Gu L, Li P. Facile route to enzyme immobilization: Core-shell nanoenzyme particles consisting of well-defined poly(methyl methacrylate) cores and cellulase shells. Langmuir, 2008, 24(19), 11036-11042.
- Khoshnevisan K, Vakhshiteh F, Barkhi M, Baharifar H, Poor-Akbar E, Zari N, Stamatis H, Bordbar A-K. Immobilization of cellulase enzyme onto magnetic nanoparticles: Applications and recent advances. Mol. Catal., 2017, 442, 66-73.
- Khoshnevisan K, Poorakbar E, Baharifar H, Barkhi M. Recent advances of cellulase immobilization onto magnetic nanoparticles: An update review. Magnetochemistry, 2019, 5(2).
- Datta S, Christena LR, Rajaram YRS. Enzyme immobilization: An overview on techniques and support materials. 3 Biotech, 2013, 3(1), 1-9.
- Falahati M, Ma’mani L, Saboury AA, Shafiee A, Foroumadi A, Badiei AR. Aminopropyl-functionalized cubic Ia3d mesoporous silica nanoparticle as an efficient support for immobilization of superoxide dismutase. Biochim. Biophys. Acta, Proteins Proteomics, 2011, 1814(9), 1195-1202.
- Jung H-S, Moon D-S, Lee J-K. Quantitative analysis and efficient surface modification of silica nanoparticles. J. Nanomater., 2012, 2012, 593471.
- Latour RA. The Langmuir isotherm: A commonly applied but misleading approach for the analysis of protein adsorption behavior. J. Biomed. Mater. Res., Part A, 2015, 103(3), 949-958.
- Latour RA. Fundamental principles of the thermodynamics and kinetics of protein adsorption to material surfaces. Colloids Surf., B, 2020, 191, 110992.
- Roach P, Farrar D, Perry CC. Interpretation of protein adsorption: Surface-induced conformational changes. J. Am. Chem. Soc., 2005, 127(22), 8168-8173.
- Secundo F. Conformational changes of enzymes upon immobilisation. Chem. Soc. Rev., 2013, 42(15), 6250-6261.
- Saha B, Saikia J, Das G. Correlating enzyme density, conformation and activity on nanoparticle surfaces in highly functional bio-nanocomposites. Analyst, 2015, 140(2), 532-542.
- Rabe M, Verdes D, Seeger S. Understanding protein adsorption phenomena at solid surfaces. Adv. Colloid Interface Sci., 2011, 162(1), 87-106.
- Sun Y, Kunc F, Balhara V, Coleman B, Kodra O, Raza M, Chen M, Brinkmann A, Lopinski GP, Johnston LJ. Quantification of amine functional groups on silica nanoparticles: A multi-method approach. Nanoscale Adv., 2019, 1(4), 1598-1607.
- Vandenberg ET, Bertilsson L, Liedberg B, Uvdal K, Erlandsson R, Elwing H, Lundström I. Structure of 3-aminopropyl triethoxy silane on silicon oxide. J. Colloid Interface Sci., 1991, 147(1), 103-118.
- Vertegel AA, Siegel RW, Dordick JS. Silica nanoparticle size influences the structure and enzymatic activity of adsorbed lysozyme. Langmuir, 2004, 20(16), 6800-6807.
- Bastida A, Sabuquillo P, Armisen P, Fernandez-Lafuente R, Huguet J, Guisan JMA. Single step purification, immobilization, and hyperactivation of lipases via interfacial adsorption on strongly hydrophobic supports. Biotechnol. Bioeng., 1998, 58(5), 486-493.
|